The open volume of MOFs can be thought of as polyhedra with metal clusters at the vertices and organic linkers along the edges. Figure 1 illustrates this concept with a cubic pore formed in MOF-5 (##Li1999).
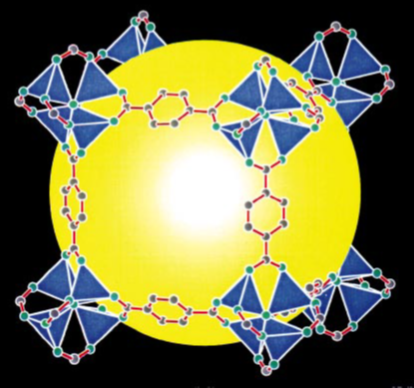
Figure 1. Crystal structure of MOF-5 with metal clusters (blue) at the corners of a cube and organic linkers at the edges of a cube (1).
One method to increase the porosity and surface area of MOFs is to increase the length of the linker between the metal vertices. However, efforts to do so often suffer from framework collapse due to a lack of linker rigidity. Interpenetration, or the growth of two lattices within one another, also tends to occur, yielding a relatively nonporous structure (2).
In a recent paper by Furukawa in the Yaghi group (3), these two failure modes for linker expansion were overcome by combining extended linkers with proper topology selection. A previously synthesized MOF, MOF-177, was expanded to yield MOF-180 and MOF-200, which exhibit 89 and 90 percent porosity, respectively (see Figure 2 below). Surface area measurements via Brunauer-Emmett-Teller (BET) adsorption were not available for MOF-180, but MOF-200 possessed a specific surface area of 4530 m2/g.
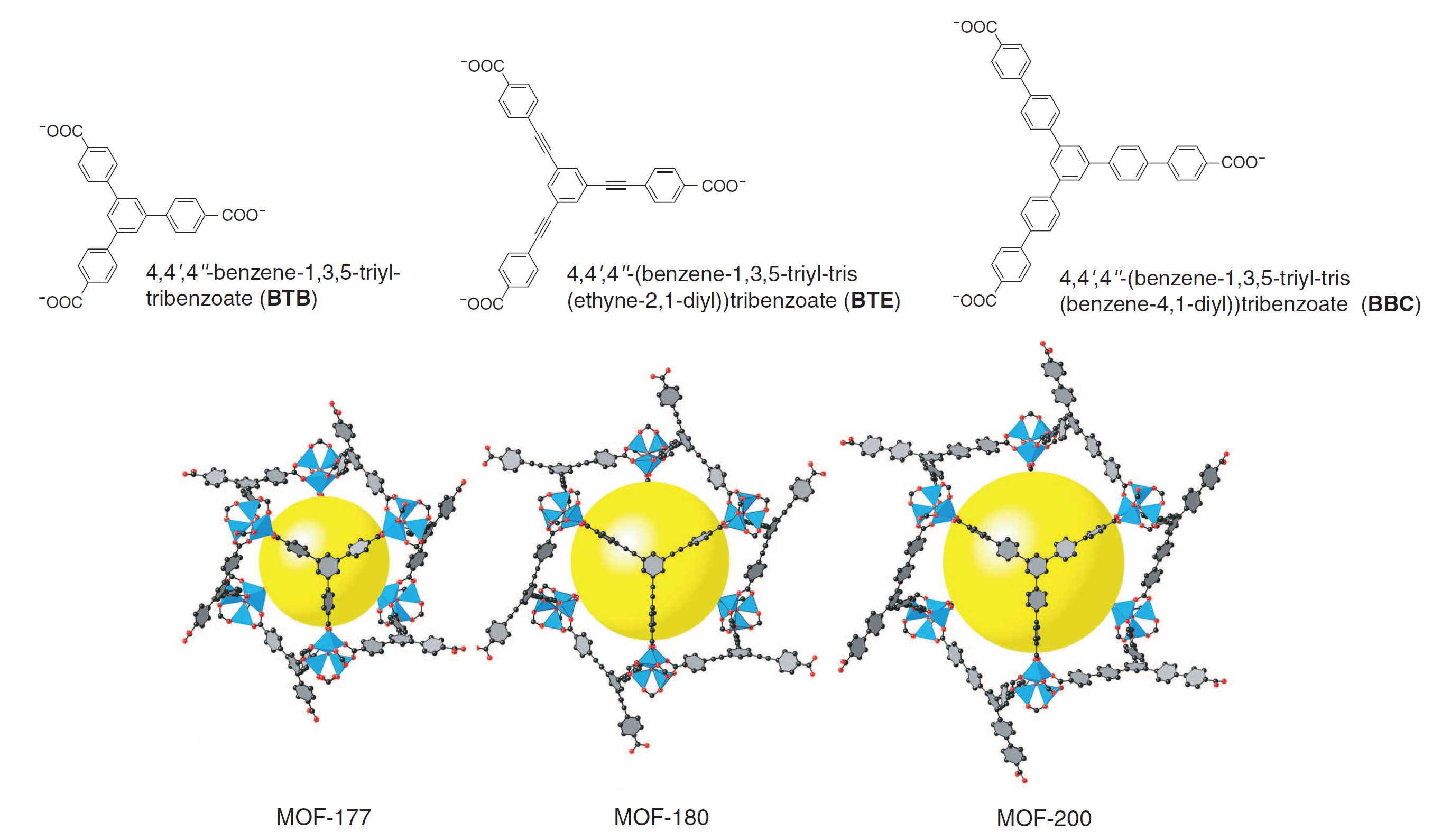
Figure 2. Crystal structures showing the expansion from MOF-177 (left) to MOF-180 (middle) and MOF-200 (right) with associated linkers above. Note the lengthening of the linkers and the increased size of the yellow sphere representing free pore space (3).
All of the above MOFs contain a single type of linker to join the metal clusters. Mixing two different types of linkers allows for additional topologies, and this idea was explored in the formation of MOF-210 from a mixture of two linkers. MOF-210 (see Figure 3) displays a completely new topology while exhibiting exceptional porosity (89%) and surface area (6240 m2/g).
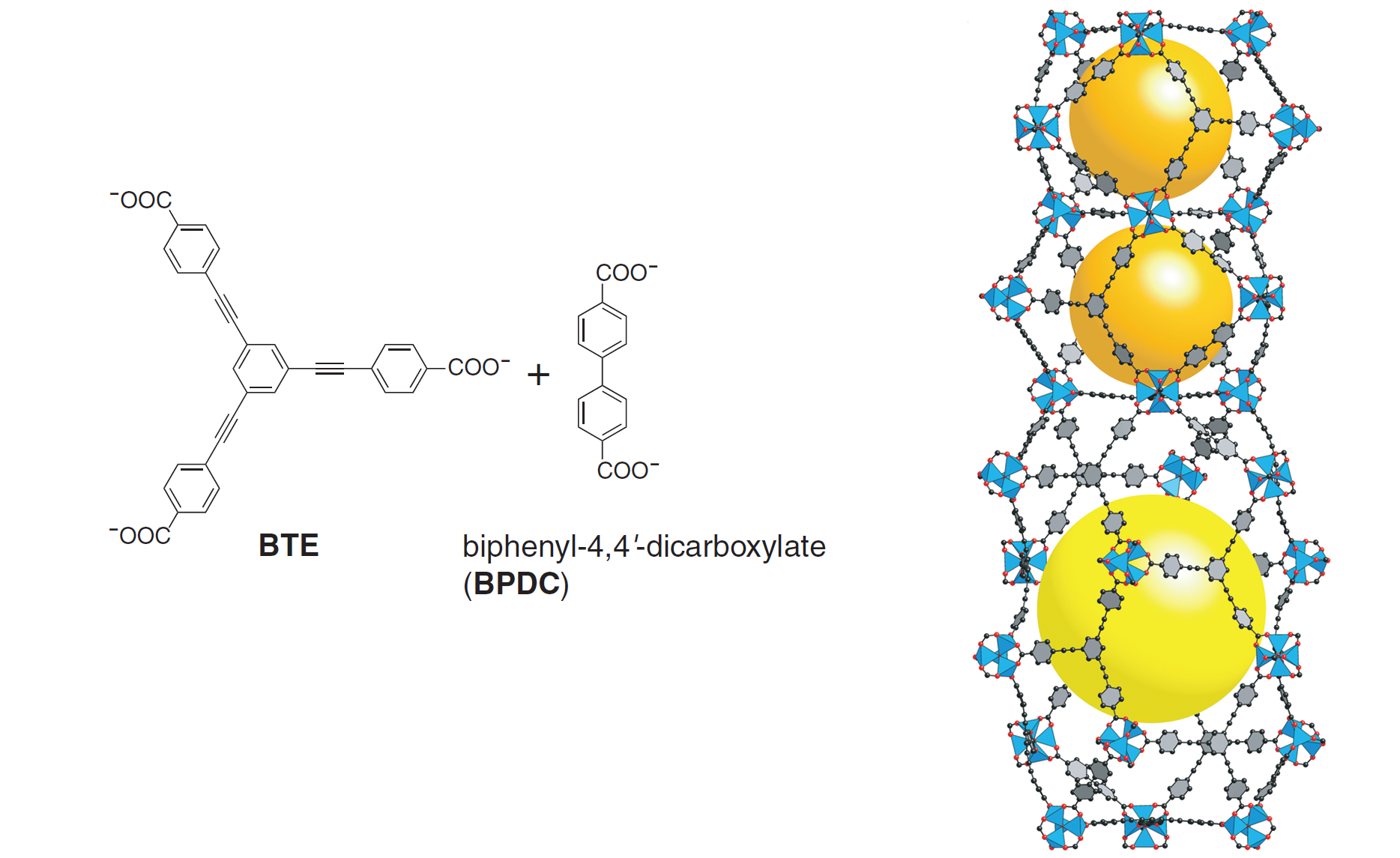
Figure 3. The two different linkers used in MOF-210 (left) and the crystal structure of MOF-210 displaying its unique topology with two different types of pores (right)
The properties of all of these MOFs far exceed conventional porous materials such as zeolites, which have on the order of 100 m2/g. In fact, MOF-210 the record for the highest specific surface area of any porous material (4). To test the performance of these materials for CO2 adsorption, the CO2 uptake was measured at room temperature and high pressure. Figure 4 shows the isotherms for five MOFs including MOF-210.
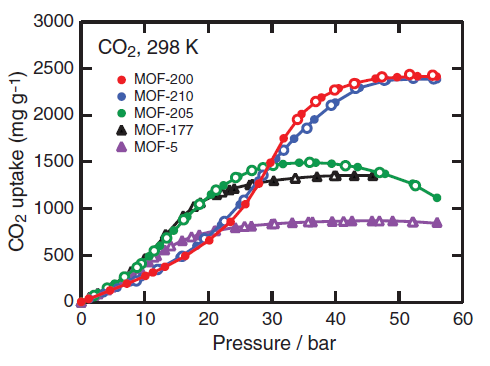
Figure 4. CO2 adsorption isotherms, including that for MOF-210. Note that MOF-210 only attains its full capacity at high CO2 pressures (3)
Despite possessing the highest surface area and porosity of MOF-210, a very high CO2 pressure of ~50 bar is necessary to saturate the material. At low pressures, which is the area of concern for CO2 capture, MOF-210 lags behind, indicating that additional work is needed to optimize capacity and low-pressure uptake.
To improve MOFs for CO2 capture, many efforts have focused on developing MOF’s that chemically adsorb (chemisorb) CO2. This increases both low-pressure capacity and the selectivity of adsorption for CO2 over other flue gas constituents, reducing the amount of adsorbent needed for a given separation. Chemisorbing MOFs can be made by including functional groups on the linkers to yield a MOF with functionalized pore walls. Alternatively, the metal centers can be made functional by using solvent molecules as coordination ligands during synthesis and subsequently removing the solvent to yield open metal coordination sites. These coordination sites can be functional themselves, or functional ligands can be attached after crystallization of the MOF. McDonald et al. used the former approach adding N,N’-dimethylethylenediamine (mmen) to exposed metal sites of CuBTTri (5). A crystal structure/schematic of the functionalized pores is shown in Figure 5.
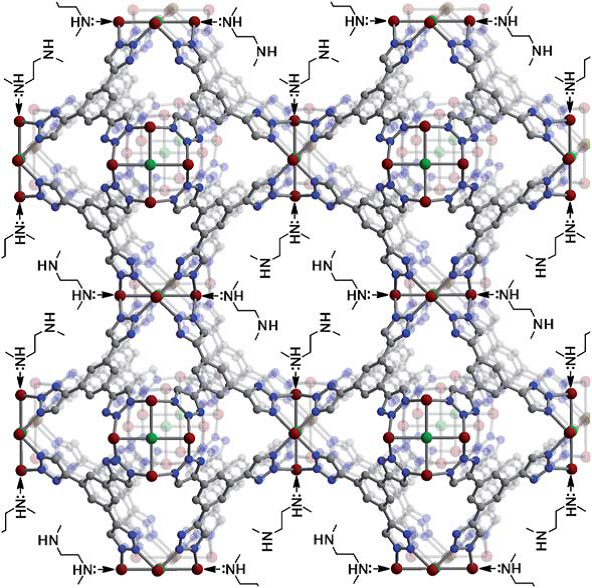
Without amine functionalization, CuBTTri exhibited a specific surface area of 1770 m2/g and an uptake of 2.9 wt% CO2 (0.15 bar of CO2 and 25°C). Note that the pressure of CO2 mimics the CO2 partial pressure of a typical flue gas stream. After amine functionalization, the specific surface area was reduced to 870 m2/g, most likely due to the reduction of pore space by mmen. However, CO2 uptake increased to 9.5 wt% (0.15 bar of CO2 and 25°C). Selectivity was modeled using a theoretical mixture of 0.15 bar CO2 and 0.75 bar of N2, and CuBTTri displayed the highest room-temperature selectivity for CO2 (327) of any MOF, as well as a very large heat of adsorption. However, selectivity decreases to 123 at typical flue gas temperatures (45°C). This large change in selectivity is advantageous for a temperature swing adsorption (TSA) process, as adsorption of CO2 decreases greatly with only a small temperature change, but cooling the flue gas to 25°C before the TSA unit would be undesirable. Further work with MOFs aim to tailor surface area, selectivity, and heats of adsorption for CO2 capture as well as study the effects of other flue gas constituents, such as water, on MOF performance.